Audio Explanation by Sir Prateek Sinha
Video Explaination
For detailed video explanation go to Ontesta Mini
Before delving into the specifics of cellular respiration, it’s crucial to establish the fundamental importance of energy in the context of living organisms. Energy is the driving force behind every biological process, from the simplest cellular functions to the most complex activities of multicellular organisms. Living organisms require energy to maintain structure, carry out metabolic processes, grow, and respond to their environment.
Imagine your body as a highly sophisticated machine that constantly performs a myriad of tasks, such as synthesizing molecules, transporting nutrients, contracting muscles, and maintaining temperature. All these activities demand a continuous and substantial supply of energy. This requirement is fulfilled by cellular respiration.
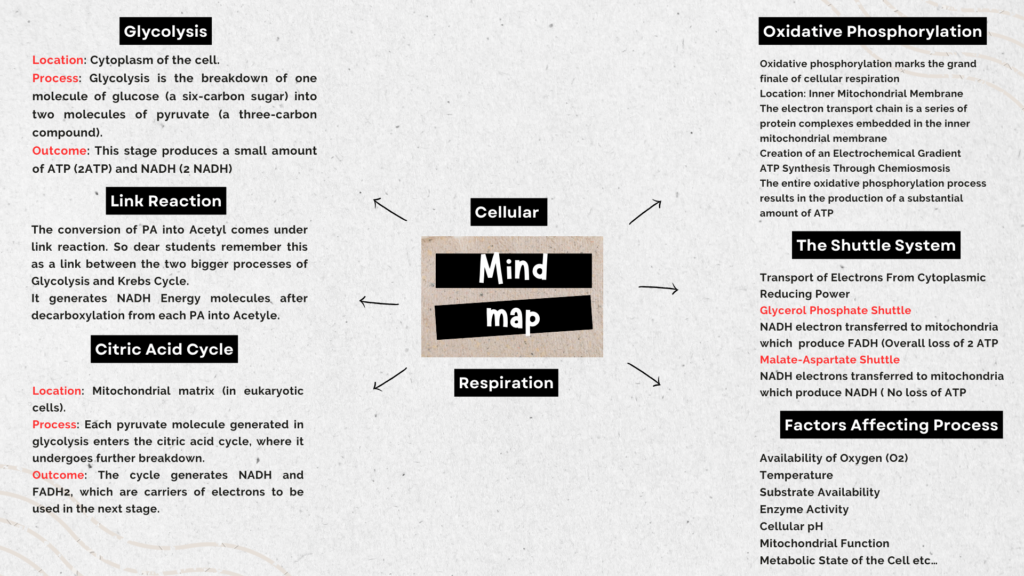
Concept
Cellular respiration is a fundamental biological process that occurs within the cells of living organisms, serving as the primary mechanism for obtaining energy from organic molecules. The primary goal of cellular respiration is to convert the chemical energy stored in nutrients, typically glucose, into a more usable and accessible form of energy known as adenosine triphosphate (ATP). ATP acts as the energy currency for cells, fueling various cellular activities and processes.1
ATP serves as the energy currency of cells, providing the necessary fuel for various cellular activities. Without an efficient energy production system, cells wouldn’t have the resources to carry out essential functions, ultimately compromising the survival and well-being of the entire organism.
The process of cellular respiration involves several interconnected stages, often occurring within different cellular compartments. The three main stages are glycolysis, the citric acid cycle (or Krebs cycle), and oxidative phosphorylation (including the electron transport chain and chemiosmosis).
Cellular respiration to daily activities and the energy needed for various functions in the body
Note : By relating cellular respiration to these daily activities, dear students you can understand how the energy derived from the process is essential for the body’s continuous functioning and adaptation to various situations. It emphasizes the dynamic relationship between the molecular processes occurring within cells and the observable activities of everyday life.
– Sir Prateek Sinha
- Physical Exercise:
- Connection to Cellular Respiration: When you engage in physical exercise, your muscles require a significant amount of energy to contract and perform work. This energy is supplied by the ATP produced through cellular respiration.
- Process Explanation: As you start exercising, your body demands more oxygen to support increased energy needs. This oxygen is essential for the final stages of cellular respiration, where the majority of ATP is generated. The increased breathing rate and heart rate during exercise ensure that cells receive sufficient oxygen for efficient energy production.
- Digestion and Nutrient Absorption:
- Connection to Cellular Respiration: After consuming a meal, the nutrients (including carbohydrates, fats, and proteins) need to be broken down and converted into a form that can be used for energy production in cellular respiration.
- Process Explanation: The digestive system breaks down complex food molecules into simpler forms. Glucose, derived from carbohydrates, is a primary substrate for cellular respiration. Nutrients absorbed in the small intestine are transported to cells, where they undergo glycolysis and enter the citric acid cycle to produce energy.
- Maintaining Body Temperature:
- Connection to Cellular Respiration: The body needs energy to maintain a constant internal temperature, especially in cold environments.
- Process Explanation: Cellular respiration contributes to the generation of heat as a byproduct. In cold conditions, the body may increase cellular respiration to produce more ATP and, in turn, more heat. This helps regulate body temperature and prevent hypothermia.
- Brain Function:
- Connection to Cellular Respiration: The brain is a highly energy-demanding organ, requiring a substantial amount of ATP to support cognitive functions.
- Process Explanation: Even during rest or mental activities, the brain relies on a continuous supply of energy from cellular respiration. Glucose is a crucial fuel for brain cells, and disruptions in this energy supply can affect cognitive performance.
- Everyday Movements:
- Connection to Cellular Respiration: Simple actions like walking, talking, and even sitting require energy that is derived from cellular respiration.
- Process Explanation: Muscle contractions, vocal cord movements, and basic bodily functions involve the utilization of ATP produced through glycolysis, the citric acid cycle, and oxidative phosphorylation.
Here’s an overview of these stages
- Glycolysis:
- Location: Cytoplasm of the cell.
- Process: Glycolysis is the breakdown of one molecule of glucose (a six-carbon sugar) into two molecules of pyruvate (a three-carbon compound).
- Outcome: This stage produces a small amount of ATP and NADH.
- Citric Acid Cycle (Krebs Cycle):
- Location: Mitochondrial matrix (in eukaryotic cells).
- Process: Each pyruvate molecule generated in glycolysis enters the citric acid cycle, where it undergoes further breakdown.
- Outcome: The cycle generates NADH and FADH2, which are carriers of electrons to be used in the next stage.
- Oxidative Phosphorylation (Electron Transport Chain and ATP Synthesis):
- Location: Inner mitochondrial membrane (in eukaryotic cells) or the plasma membrane (in prokaryotic cells).
- Process: Electrons carried by NADH and FADH2 from glycolysis and the citric acid cycle are transferred through a series of protein complexes in the electron transport chain. This creates an electrochemical gradient, driving ATP synthesis through chemiosmosis.
- Outcome: The majority of ATP is produced in this stage
The overall chemical equation for cellular respiration is

Glycolysis: Cellular Respiration Kickstart
Glycolysis is the initial stage of cellular respiration, and it takes place in the cytoplasm of the cell. This process serves as the entry point for the catabolism of glucose, a six-carbon sugar, into smaller, more manageable compounds. Let’s break down the key aspects of glycolysis:
Location: Cytoplasm
- Glycolysis occurs in the cytoplasm, the gel-like substance that fills the interior of the cell. Unlike the later stages of cellular respiration, which occur in the mitochondria, glycolysis happens in a cellular compartment that is more accessible and does not require the presence of specialized organelles.
Starting Point: One Molecule of Glucose
- The process begins with one molecule of glucose, a primary source of energy for cellular activities. Glucose is a six-carbon sugar that enters the cell through various transport mechanisms.
Series of Reactions:
Glycolysis involves a sequence of ten enzymatic reactions. These reactions systematically transform one molecule of glucose into two molecules of pyruvate, a three-carbon compound. Each step of glycolysis is catalyzed by specific enzymes.
Investment and Payoff Phases:
- Glycolysis can be divided into two phases: the energy-investment phase and the energy-payoff phase.
- Energy-Investment Phase: This initial phase consumes ATP to activate glucose and prepare it for further breakdown. It involves reactions that phosphorylate and rearrange the glucose molecule.
- Energy-Payoff Phase: In this phase, the activated glucose undergoes a series of reactions, leading to the production of ATP and NADH (Nicotinamide Adenine Dinucleotide). ATP is both consumed and produced during glycolysis, resulting in a net gain of ATP molecules.
Formation of Pyruvate:
- The final steps of glycolysis involve the cleavage of a six-carbon sugar (fructose-1,6-bisphosphate) into two molecules of three-carbon compounds, each resembling pyruvate.
- This step also yields ATP and NADH as byproducts.
End Product: Two Molecules of Pyruvate
- At the end of glycolysis, one molecule of glucose is transformed into two molecules of pyruvate. These pyruvate molecules are then ready to enter the next stage of cellular respiration, the citric acid cycle, where further breakdown and energy extraction will occur.
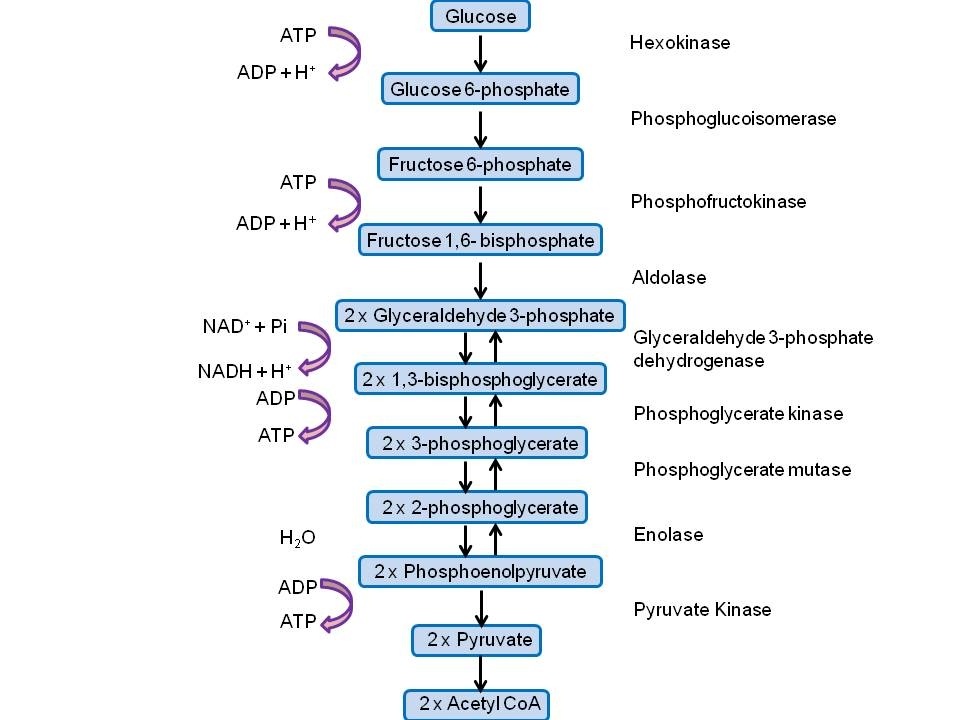
Note : The conversion of PA into Acetyl comes under link reaction. So dear students remember this as a link between the two bigger processes of Glycolysis and Krebs Cycle.
– Sir Prateek Sinha
The link reaction, also known as the pyruvate dehydrogenase complex, is a crucial step that connects glycolysis to the citric acid cycle in cellular respiration. In this process:
Location – Mitochondrial Matrix
- Starting Point: Pyruvate from Glycolysis
- Pyruvate, the three-carbon end product of glycolysis, enters the mitochondria, where the link reaction occurs.
- Conversion to Acetyl-CoA:
- Each pyruvate molecule undergoes decarboxylation and loses a carbon dioxide (CO2). This reaction results in the formation of acetyl coenzyme A (acetyl-CoA), a two-carbon compound.
- NADH Production:
- During the decarboxylation, NAD+ is reduced to NADH. This electron carrier will play a role in the subsequent stages of cellular respiration.
- Connection to the Citric Acid Cycle:
- Acetyl-CoA, the product of the link reaction, serves as the precursor for the citric acid cycle. It enters the cycle and combines with oxaloacetate to initiate the series of reactions that extract more energy from the carbon compounds.
In essence, the link reaction prepares the products of glycolysis (pyruvate) for further energy extraction in the citric acid cycle by converting them into acetyl-CoA. It also generates NADH, which becomes a vital player in the subsequent stages of cellular respiration. The link reaction is a bridge between glycolysis and the citric acid cycle, ensuring the smooth continuation of the energy-yielding process in the mitochondria.
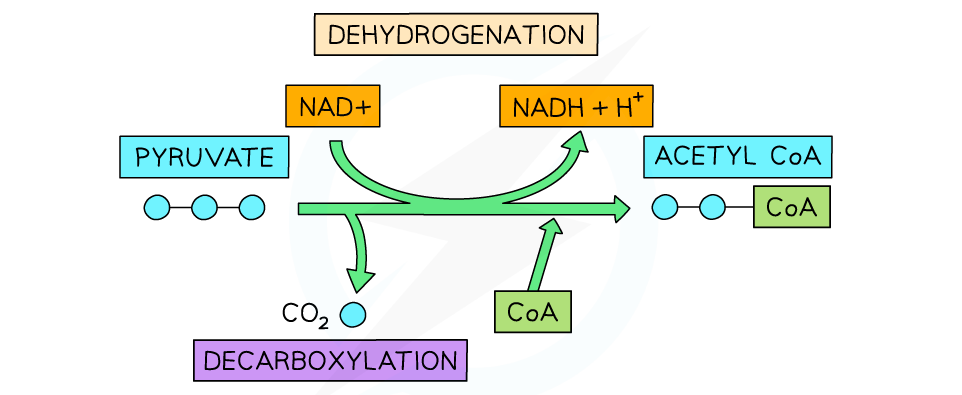
The Citric Acid Cycle
The citric acid cycle, also known as the Krebs cycle, is the second stage of cellular respiration, taking place within the mitochondrial matrix. This cycle is a pivotal component in the process of extracting energy from glucose. Let’s explore the key features of the citric acid cycle:
- Location: Mitochondrial Matrix
- The mitochondrial matrix, the innermost compartment of the mitochondria, serves as the site for the citric acid cycle. This location allows for the seclusion and efficient control of the cycle within the cell.
- Starting Point: Acetyl-CoA Enters the Cycle
- Acetyl coenzyme A (acetyl-CoA), the product of the link reaction, enters the citric acid cycle. Each acetyl-CoA is derived from the breakdown of one molecule of pyruvate.
- Combination with Oxaloacetate:
- The acetyl group from acetyl-CoA combines with oxaloacetate, a four-carbon compound present in the cycle, forming citrate, a six-carbon compound.
- Series of Reactions:
- The citric acid cycle consists of a series of enzymatic reactions that systematically break down citrate, releasing carbon dioxide and generating energy-rich molecules.
- Energy Production:
- Throughout the cycle, electrons are transferred to carrier molecules, generating NADH and FADH2. These electron carriers play a crucial role in the subsequent stage of cellular respiration, the oxidative phosphorylation.
- Regeneration of Oxaloacetate:
- Oxaloacetate is regenerated at the end of each cycle, ready to combine with another acetyl group from acetyl-CoA in the next round.
- Carbon Dioxide Release:
- Each turn of the citric acid cycle results in the release of two carbon dioxide molecules. These CO2 molecules represent the carbon atoms originally present in the acetyl-CoA.
- Net Outcome per Acetyl-CoA:
- For every acetyl-CoA entering the cycle, three NADH molecules, one FADH2 molecule, and one ATP (or GTP, a molecule with similar energy-carrying capacity) are produced. The cycle repeats for each pyruvate molecule generated during glycolysis.
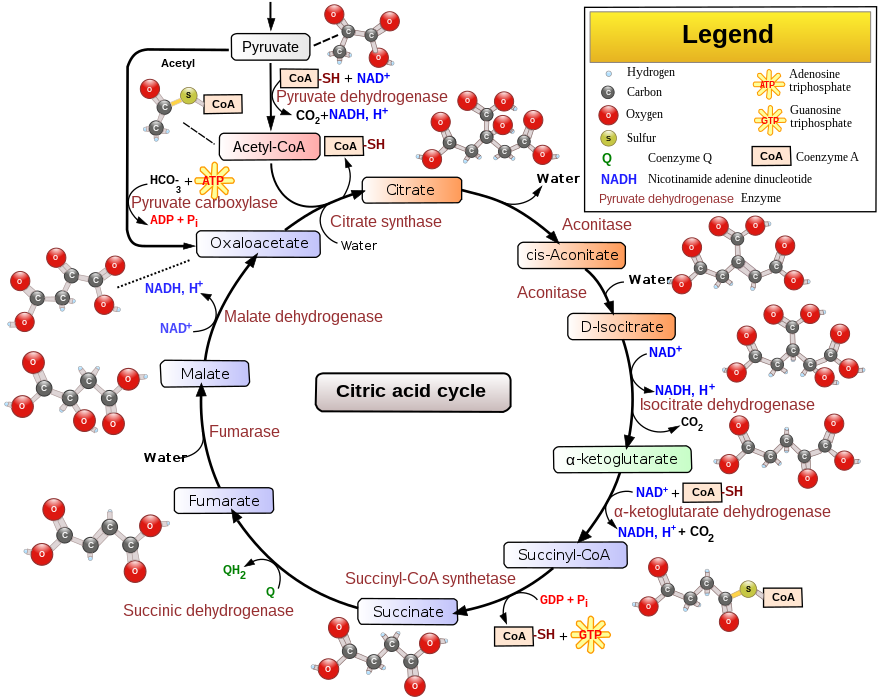
Source of above Image: Wikipedia
Oxidative Phosphorylation:
Unleashing the Power of Electron Transport
Oxidative phosphorylation marks the grand finale of cellular respiration, taking place in the inner mitochondrial membrane. This complex and highly efficient stage culminates in the production of the majority of adenosine triphosphate (ATP), the cell’s primary energy currency. Let’s dive into the intricacies of oxidative phosphorylation:
- Location: Inner Mitochondrial Membrane
- Oxidative phosphorylation occurs in the inner mitochondrial membrane, a strategically positioned site where the flow of electrons and the synthesis of ATP are tightly coupled.
- Electron Transport Chain (ETC):
- The electron transport chain is a series of protein complexes embedded in the inner mitochondrial membrane. It consists of proteins with varying affinities for electrons, creating a dynamic pathway for electron flow.
- Movement of Electrons:
- High-energy electrons derived from NADH and FADH2, products of glycolysis and the citric acid cycle, are shuttled through the protein complexes of the electron transport chain. As electrons move along this chain, they release energy.
- Creation of an Electrochemical Gradient:
- The energy released by the movement of electrons is used to pump protons (H+ ions) across the inner mitochondrial membrane, creating an electrochemical gradient. This process establishes a higher concentration of protons in the intermembrane space compared to the mitochondrial matrix.
- ATP Synthesis Through Chemiosmosis:
- The electrochemical gradient serves as the driving force for ATP synthesis through a process called chemiosmosis. The potential energy stored in the form of this gradient is harnessed to produce ATP.
- Protons move back into the mitochondrial matrix through a specialized enzyme called ATP synthase, which is embedded in the inner mitochondrial membrane. This movement of protons through ATP synthase induces a conformational change in the enzyme, facilitating the synthesis of ATP from adenosine diphosphate (ADP) and inorganic phosphate (Pi).
- Role of Oxygen:
- Oxygen plays a crucial role as the final electron acceptor in the electron transport chain. It combines with electrons and protons to form water in a process known as the reduction of oxygen. This ensures the continuous flow of electrons through the chain.
- Net Result: ATP Production:
- The entire oxidative phosphorylation process results in the production of a substantial amount of ATP. The exact yield depends on the number of NADH and FADH2 molecules generated during glycolysis and the citric acid cycle.
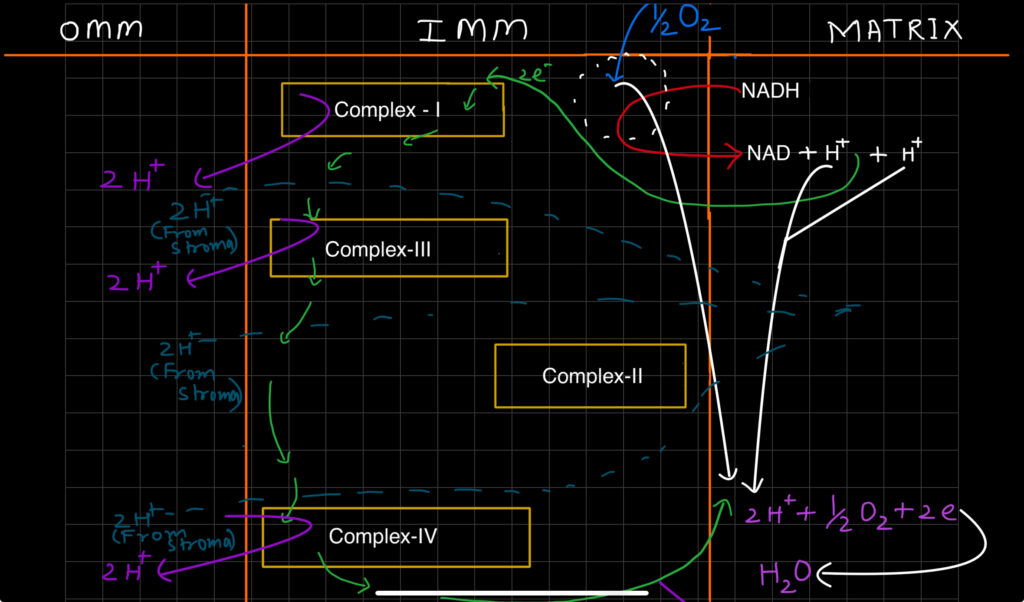

Overview
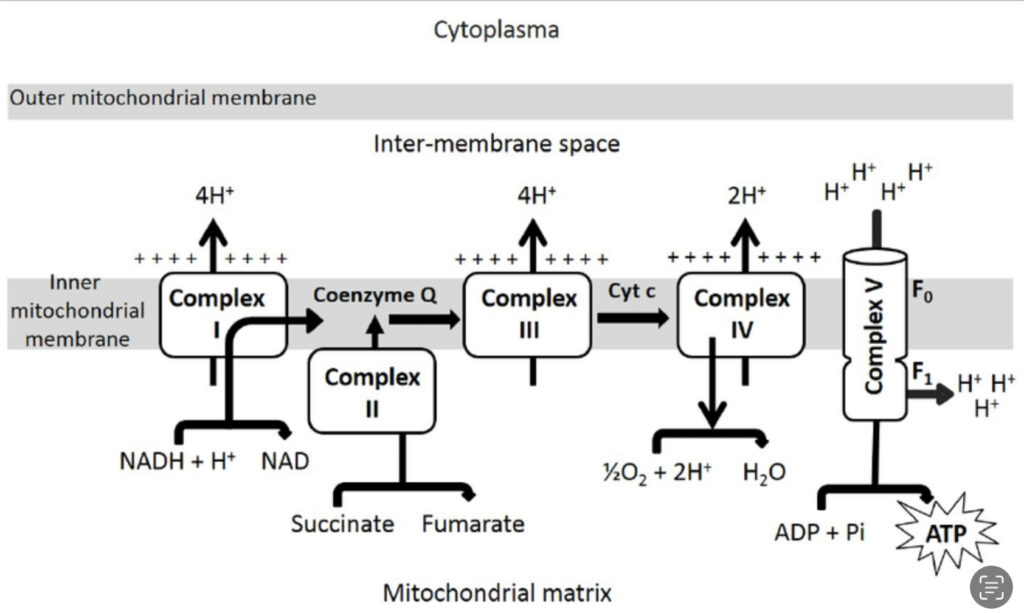
The Shuttle System (Transport of Electrons From Cytoplasmic Reducing Power)
- Glycerol Phosphate Shuttle:
- Overview: The glycerol phosphate shuttle is a mechanism by which electrons are transferred from cytoplasmic NADH to the mitochondrial electron transport chain (ETC), facilitating ATP production.
- Steps:
- In the cytoplasm, glycerol 3-phosphate dehydrogenase converts cytoplasmic NADH to glycerol 3-phosphate.
- Glycerol 3-phosphate can then enter the mitochondria, where glycerol 3-phosphate dehydrogenase on the inner mitochondrial membrane converts it back to dihydroxyacetone phosphate, generating mitochondrial FADH2.
- FADH2 enters the electron transport chain at a lower energy level than NADH, leading to the production of fewer ATP molecules during oxidative phosphorylation.
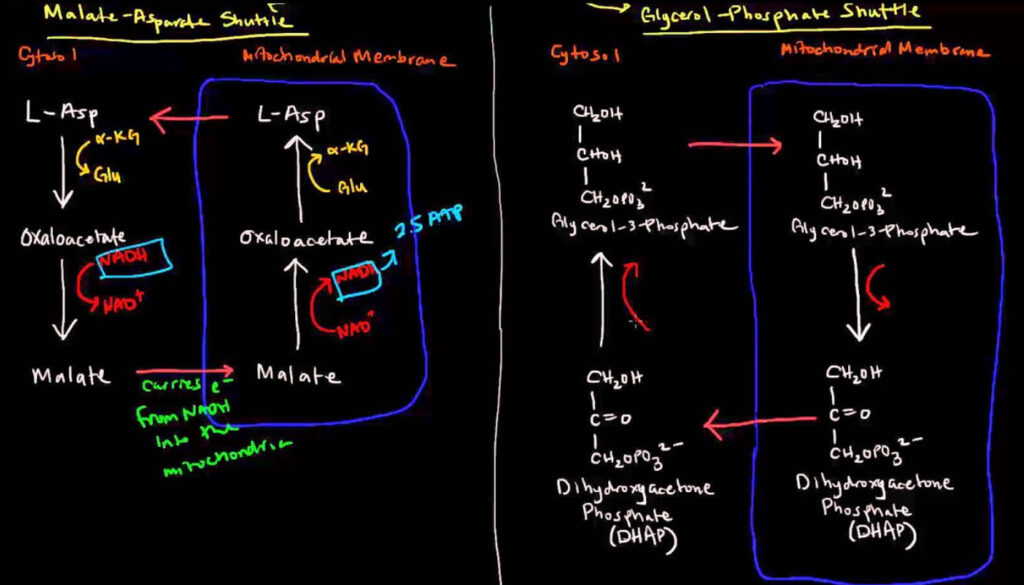
- Malate-Aspartate Shuttle:
- Overview: The malate-aspartate shuttle is another mechanism for transferring electrons from the cytoplasm to the mitochondria, allowing NADH generated in the cytoplasm to contribute to ATP production in the mitochondria.
- Steps:
- In the cytoplasm, oxaloacetate (OAA) is reduced to malate by cytoplasmic malate dehydrogenase, converting cytoplasmic NADH to NAD+.
- Malate can then move into the mitochondria.
- Once in the mitochondria, malate is oxidized back to oxaloacetate by mitochondrial malate dehydrogenase, reducing mitochondrial NAD+ to NADH.
- Oxaloacetate is then transaminated to aspartate, which can exit the mitochondria.
- In the cytoplasm, aspartate is transaminated back to oxaloacetate, completing the cycle.
Both shuttle systems play crucial roles in maintaining the balance of reducing equivalents and energy flow between the cytoplasm and mitochondria, ensuring efficient ATP production during cellular respiration.
Factors that Affects Cellular Respiration
- Availability of Oxygen (O2):
- Cellular respiration can be aerobic or anaerobic. Aerobic respiration, which occurs in the presence of oxygen, is more efficient in generating ATP compared to anaerobic respiration. The availability of oxygen influences the type and efficiency of respiration.
- Temperature:
- Cellular respiration rates are affected by temperature. Generally, an increase in temperature leads to an increase in the rate of chemical reactions, including those involved in cellular respiration. However, extremely high temperatures can denature enzymes and disrupt cellular processes.
- Substrate Availability:
- The availability of substrates, such as glucose and other organic molecules, directly impacts cellular respiration. The type and amount of substrate molecules determine the fuel source for respiration and influence the overall energy production.
- Enzyme Activity:
- Enzymes play a crucial role in catalyzing the various reactions involved in cellular respiration. Factors such as pH, temperature, and the presence of cofactors can affect enzyme activity and, consequently, impact the efficiency of respiration.
- Cellular pH:
- The pH of the cellular environment influences enzyme activity and protein structure. Cellular respiration involves multiple enzymatic reactions, and maintaining an optimal pH is essential for their proper functioning.
- Mitochondrial Function:
- The health and efficiency of mitochondria, the cellular organelles responsible for respiration, are critical for overall cellular respiration. Factors such as the number of mitochondria, their structural integrity, and the presence of necessary cofactors influence respiration.
- Metabolic State of the Cell:
- The metabolic state of a cell, including factors such as the energy demands, availability of nutrients, and the presence of signaling molecules, can regulate the rate of cellular respiration. Cells adjust their respiration rates to meet their energy requirements.
- Hormones and Signaling Molecules:
- Hormones and other signaling molecules can influence cellular respiration by regulating the expression of genes involved in metabolic pathways. For example, insulin promotes glucose uptake, affecting the availability of substrate for respiration.
- Cell Type and Differentiation:
- Different cell types may have varying energy demands, and the differentiation state of cells can influence their metabolic activity. Specialized cells may exhibit unique respiratory characteristics based on their functions.
Understanding these factors is crucial for appreciating the dynamic nature of cellular respiration and its adaptation to changing cellular conditions. The intricate interplay of these factors ensures that cells can efficiently produce energy to meet their specific needs.
Additional Factors
- Nutrient Availability:
- Cellular respiration relies on the availability of nutrients, including carbohydrates, fats, and proteins. The breakdown of these macromolecules provides the necessary substrates for various stages of respiration. Changes in nutrient availability, such as fasting or a high-fat diet, can impact the type and efficiency of cellular respiration.
- Energy Demand and Cellular Activity:
- Cells adjust their respiratory rates based on their energy demands. Highly active cells or tissues, such as muscle cells during exercise, may undergo increased respiration to meet elevated ATP requirements. Conversely, cells at rest may exhibit lower respiratory rates.
- Genetic Factors:
- Genetic factors influence the expression of genes involved in cellular respiration. Variations in genes encoding enzymes and proteins associated with respiration can affect the efficiency of the process and contribute to individual variations in energy metabolism.
- Disease and Pathological Conditions:
- Various diseases and pathological conditions can impact cellular respiration. For example, mitochondrial disorders affect the function of mitochondria, leading to impaired respiration and reduced ATP production. Conditions like diabetes can alter the utilization of glucose in respiration.
- Redox State:
- Cellular respiration involves redox reactions, where electrons are transferred between molecules. The redox state, determined by the balance of oxidized and reduced molecules, affects the flow of electrons through the electron transport chain. Disruptions in the redox balance can impact respiration.
- Cellular Stress and Environmental Factors:
- Stressful conditions, such as exposure to toxins, radiation, or extreme environmental factors, can influence cellular respiration. Cells may adapt their metabolic activities in response to external stressors to maintain energy homeostasis and cell survival.
- Age:
- Cellular respiration can be influenced by age-related changes in cells and tissues. Mitochondrial function may decline with age, affecting the overall efficiency of cellular respiration. This can contribute to the aging process and age-related diseases.
- Oxidative Stress:
- The production of reactive oxygen species (ROS) during cellular respiration can lead to oxidative stress. Excessive ROS production, often associated with mitochondrial dysfunction, can damage cellular components and impact overall cellular health.
Understanding the multifaceted nature of the factors affecting cellular respiration is essential for comprehending how cells regulate their energy production in response to internal and external cues. Cells employ intricate regulatory mechanisms to optimize energy metabolism, ensuring survival and proper functioning in diverse physiological conditions
Suggested Books/Reviews/Research/Journals/Advancements
Certainly! Below is a list of references that cover various aspects of cellular respiration. Please note that specific references may depend on your area of interest within cellular respiration, and it’s always a good idea to search for the most recent and relevant literature in academic databases.
- Books:
- Alberts, B., Johnson, A., Lewis, J., Morgan, D., Raff, M., Roberts, K., & Walter, P. (2014). “Molecular Biology of the Cell” (6th ed.). Garland Science.
- Nelson, D. L., & Cox, M. M. (2017). “Lehninger Principles of Biochemistry” (7th ed.). W. H. Freeman.
- Berg, J. M., Tymoczko, J. L., & Stryer, L. (2019). “Biochemistry” (8th ed.). W. H. Freeman.
- Review Articles:
- Wallace, D. C. (2005). “A mitochondrial paradigm of metabolic and degenerative diseases, aging, and cancer: a dawn for evolutionary medicine.” Annual Review of Genetics, 39, 359-407.
- Brand, M. D., & Nicholls, D. G. (2011). “Assessing mitochondrial dysfunction in cells.” Biochemical Journal, 435(2), 297-312.
- Murphy, M. P. (2009). “How mitochondria produce reactive oxygen species.” Biochemical Journal, 417(1), 1-13.
- Research Papers:
- Chance, B., & Williams, G. R. (1955). “The respiratory chain and oxidative phosphorylation.” Advances in Enzymology and Related Subjects of Biochemistry, 17, 65-134.
- Mitchell, P. (1961). “Coupling of phosphorylation to electron and hydrogen transfer by a chemi-osmotic type of mechanism.” Nature, 191(4784), 144-148.
- Wallace, D. C. (1999). “Mitochondrial diseases in man and mouse.” Science, 283(5407), 1482-1488.
- Journal Articles:
- Chandel, N. S. (2015). “Mitochondria as signaling organelles.” BMC Biology, 13(1), 34.
- Nicholls, D. G., & Ferguson, S. J. (2002). “Bioenergetics 3.” Academic Press.
- Recent Advances:
- Rizzuto, R., De Stefani, D., Raffaello, A., & Mammucari, C. (2012). “Mitochondria as sensors and regulators of calcium signalling.” Nature Reviews Molecular Cell Biology, 13(9), 566-578.
- Cogliati, S., Frezza, C., Soriano, M. E., Varanita, T., Quintana-Cabrera, R., Corrado, M., … & Scorrano, L. (2013). “Mitochondrial cristae shape determines respiratory chain supercomplexes assembly and respiratory efficiency.” Cell, 155(1), 160-171.
Research Journals:
- Cell Metabolism
- Journal of Biological Chemistry (JBC)
- Nature Reviews Molecular Cell Biology
- Trends in Biochemical Sciences
- Annual Review of Biochemistry
- Biochimica et Biophysica Acta (BBA) – Bioenergetics
Really helpful , this makes the concept more clearer in minds . Very informative 👍👍
Thank you! We are here to help you to understand each and every concept like this.
Keep Supporting us
– Study Buddy Shareline
Ontesta Family
Ye bahut achcha likha hai ….heads off
We need more such content
Thanks Aarushi